Thank you for visiting nature.com. You are using a browser version with limited support for CSS. To obtain the best experience, we recommend you use a more up to date browser (or turn off compatibility mode in Internet Explorer). In the meantime, to ensure continued support, we are displaying the site without styles and JavaScript.
Scientific Reports volume 12, Article number: 11059 (2022 ) Cite this article Ho2O3
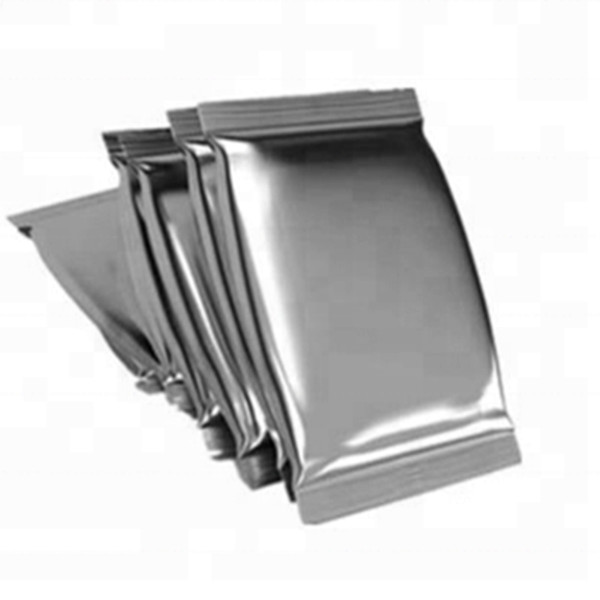
The effect of CeF3 concentration and γ-irradiation on the physical, optical and luminescence properties of Gd2O3–B2O3–CeF3 glasses were studied in this work. Before irradiation, the addition of CeF3 in glass degraded the network connectivity observed from FTIR and possibly created the non-bridging oxygen (NBO) in glass structure. This NBO caused the reduction of Ce3+/Ce4+ ratio in XANES, the red-shift in transmission spectra and the raise of refractive index with addition of CeF3 content. Such red-shift also was influenced by 4f–5d transition of Ce3+ dopant. This ion generated the strongest photoluminescence (PL) and radioluminescence (RL) in 0.3 mol% CeF3-doped glass with nanoseconds decay time. The irradiation with γ-rays damaged the glass structure, broke the chemical bonds, and created color center in the glass network. The non-bridging oxygen hole center (NBOHC), that absorbed photons in the visible light region, caused the darkening, color change and increment of refractive index. These irradiation effects on glass were mitigated by the addition of CeF3 that the electron donation of Ce3+ decreased the number of NBOHC. The Ce3+/Ce4+ ratio in most glasses after irradiation then reduced compared to them before irradiation, resulting to the decrease in PL and RL intensity. Our results confirm that CeF3 can enhance the radiation hardness of glass and the 0.3 mol% CeF3-doped glass is a promising glass scintillator.
Single crystal scintillators are used in various applications such as medical imaging, non-destructive inspection, nuclear or high energy physics, environmental monitoring and geological exploration. In radiation detectors, single crystals offer the advantage of having high light yields and fast response times1,2. However, single crystal growth is an expensive and slow process; and single crystals can only be produced with limited shapes and sizes. On the other hand, glasses with various shapes and sizes are cheaper and faster to fabricate. Recently, the glass scintillators have been developed and several works have shown sufficient high light yields and fast decay times for practical applications3,4,5, including the interaction of radiation with glass and their shielding properties6,7,8,9,10,11,12,13,14,15,16,17. Investigation of novel glasses for radiation detection is therefore emerging, with particular focus on understanding the irradiation effects and improving the radiation hardness18. The radiation hardness is the resistant of material that its properties was not changed or distorted by irradiation.
Gd2O3–B2O3-based glasses are suitable scintillators owing to their radiation interaction. The 10B boron isotope possesses a high capture cross-section for thermal neutrons, making it a suitable neutron detector19,20,21. Additionally, B2O3 host glass is highly transparent, with good physical and chemical properties that meet the requirements for a scintillator19,22,23. The high phonon energy of borate glass decreases its luminescence efficiency24, but this can be mitigated by adding a heavy metal oxide, such as Gd2O3, into the glass25,26. For γ-rays and X-rays detection, the addition of Gd increases the glass density and effective atomic number which improves the interaction between glass and such incoming radiation27,28,29 and the Gd3+ ion can efficiently transfer the energy to luminescence centers such as lanthanide ions (Ln3+)20,30,31. In case of neutron detection, the 155Gd and 157Gd isotopes own a high capture cross-section for thermal neutrons20,27,32. However, there is low energy γ-rays emitted from Gd under neutron irradiation, and distinguish this γ-rays from background γ-rays is technically impossible in the pulse height or spectroscopy-based techniques. Therefore, there is no one uses Gd-based scintillators for actual applications except for some special applications. If consider in the common pulse shape discrimination it may be possible to use33. Therefore, the Gd2O3–B2O3-based glasses is very attractive for γ-rays and X-rays scintillator, but it has a difficulty for using in neutron detection.
Among the Ln3+ ions, trivalent cerium (Ce3+) is the most favored luminescence center for scintillator applications because the 5d–4f dipole allowed transition in Ce3+ results to a bright luminescence emission with nanoseconds decay time34,35,36. Previous works have investigated the Ce3+-doped Gd2O3–B2O3-based glasses such as Ce3+: Li2O3–Bi2O3–Gd2O3–B2O337, Ce3+: Gd2O3–CaO–SiO2–B2O338, Ce3+: Li2O–Gd2O3–BaO–B2O339, Ce3+-Dy3+: CaCO3–ZnO–Gd2O3–B2O340, and Ce3+: Gd2O3–B2O341. In particular, our work on the xCeF3-doped pure binary 27.5Gd2O3–(72.5 − x)B2O3 (Ce:GB)41 demonstrated the significant progress in binary glass preparation as the glass sample was successfully synthesized without adding any glass modifier compound to help in glass melting process. Our technique has the advantage of excluding unnecessary oxide components which possibly degrade the color, optical and luminescence properties of the glass. Consequently, the Ce:GB glass exhibited characteristics that make it a promising glass scintillator.
In order to fully capitalize on the potential of Ce:GB and other glasses as scintillators, in-depth investigation about the effects of irradiation on the glass’ properties, especially on its luminescence properties and radiation-hardness, are necessary for further study. Consequently, the Ce:GB glasses were irradiated by gamma-rays (γ-rays) and the effect of this irradiation on glass properties were invein binary glass preparation as the glassstigated in this present work.
Ce:GB glasses with 27.5Gd2O3-(72.5-x)B2O3-xCeF3 composition were synthesized by a melt-quenching technique. The glass samples, 0CeGB, 0.05CeGB, 0.1CeGB, 0.3CeGB, 0.5CeGB, 1.0CeGB and 1.5CeGB contain different CeF3 concentrations, with x being 0.00, 0.05, 0.10, 0.30, 0.50, 1.00 and 1.50 mol%, respectively. Details of the raw chemicals and the glass preparation procedure are stated in our previous work41. The Ce:GB glasses were irradiated with γ-rays carrying 1.17 and 1.33 MeV energies from a cobalt-60 (60Co) source. The 60Co source was calibrated with water and had a dose rate of 36.82 Gy/h at a distance of 1.0 m. The samples were placed at approximately 10 cm away from the radiation source for 6 h. The irradiation was performed at room temperature and in ambient atmosphere. The estimated irradiation dose rate and total dose on samples are 0.57 kGy/hour and 3.44 kGy, respectively. The oxidation state of Ce ion dopant in glasses were monitored by X-ray absorption near edge structure (XANES) spectroscopy at the Synchrotron Light Research Institute (SLRI), Thailand. The glasses densities (ρ) were determined using a 4-digit microbalance (AND, HR-200) and the Archimedes’ method41 with deionized water as an immersion liquid. The molar volumes of the glasses (VM) were calculated using the relation: VM = MT/ρ. The refractive indices (n) of the glasses were measured by Abbe refractometer (Atago, DR-M2/M4) using the D-line (589 nm) source and 1-bromonapthalene as the contact liquid. Fourier-transform infrared (FTIR) spectra were recorded using an FTIR spectrometer (Agilent, Cary 630). An Ultraviolet–Visible–near infrared (UV–VIS–NIR) spectrophotometer (Shimadzu, UV-3600) was used to measure the transmittance spectra. The photoluminescence (PL) spectra of glasses were monitored by a spectrofluorophotometer (Agilent, Cary Eclipse) with xenon lamp as a light source. The PL decay profiles were obtained using the third harmonics (3ω, 290 nm) of a Ti:sapphire laser. The decay times were measured using a 25 cm focal length spectrograph which was fitted with a 600 grooves mm−1 grating that was coupled to a Hamamatsu C1587 streak camera unit and a charge-coupled device (CCD) camera. For the X-ray induced optical luminescence or radioluminescence (RL) spectra, the glass samples were excited by X-rays from a Cu target generator (Inel, XRG3D-E) with 50 kV and 30 mA power. The RL emission signal was detected by an optical fiber and a spectrometer (Ocean Optics, QE65 Pro).
Photographs showing the physical appearance of Ce:GB glasses before and after γ-ray irradiation are represented in Fig. 1. Before irradiation, the CeF3-free glass (0CeGB) was highly transparent and colorless; while the color of the CeF3-doped glasses (0.05CeGB, 0.1CeGB, 0.3CeGB, 0.5CeGB, 1.0CeGB and 1.5CeGB) became more greenish yellow as the amount of CeF3 increased. After irradiation, the 0CeGB glass was dramatically darkened and least transparent, indicating that there was significant damage from the γ-rays. On the other hand, the irradiated CeF3-doped glasses was less darkened and hence more transparent than the 0CeGB glass. A greenish yellowing in the glasses can be observed which the 1.5CeGB glass visually exhibited a similar level of transparency and color tone before and after irradiation.
The Ce:GB glasses before and after γ-irradiation.
Table 1 shows the density (ρ) and molar volume (VM) of all Ce:GB glasses before and after γ-irradiation. The density of Ce:GB glasses were quite high in a range of 4.09–4.16 g/cm3, which are suitable for radiation detection28. The CeF3 concentration and irradiation did not seem to significantly affect the density and molar volume of glasses. Generally, the density of glass decreases if glass is irradiated by huge γ-rays that ejects the anions in structure. However, that density change is very small which requires high accurate measuring system to observe and the irradiation dose using in this work was not high. The increment of density after irradiation in 0.05CeGB, 0.1CeGB and 0.5CeGB glass were lower than 1%, so they could be the typical errors from measurement.
The typical XANES spectra represent the Ce LIII edge of 0.05CeGB, 0.3CeGB and 1.5CeGB glasses before (Fig. 2a) and after irradiation (Fig. 2b), compared to the unirradiated standard compounds, CeF3 and CeO2. The XANES spectra show that the + 3-oxidation state of Ce3+ in CeF3 compound has a prominent absorption peak at 5727 eV, while the + 4-oxidation state of Ce4+ in CeO2 powder has obvious double peaks at 5731 eV and 5738 eV. By comparing both standard compounds, there was also a weak absorption peak of Ce3+ in the CeO2 powder. Likewise, there were weak peaks of Ce4+ in the CeF3 compound. These indicate that the cerium ions in CeF3 and CeO2 coexisted in both Ce3+ and Ce4+ states. The Ce:GB glasses in this work were doped with CeF3. Therefore, their XANES spectra mimiced the spectrum of CeF3 standard where the Ce3+ ion is dominant. The XANES data were evaluated using the Athena software to ascertain the quantity percentage of Ce3+ and Ce4+ ions in Ce:GB glasses. Before irradiation, the ratio of Ce3+/Ce4+ in the glasses decreased as the CeF3 concentration increased. The same trend was also observed in CaO–SiO2–B2O3–CeF3 and SiO2–Al2O3–Li2O–Na2O–K2O–BaO–SrO–Tb2O3–Gd2O3–CeO2 glass fabricated in air atmosphere by Rajaramakrishna et al.38 and Zu et al.42, respectively. On the other hand, the Ce3+/Ce4+ ratio in the glasses after irradiation increased with the increase in CeF3 concentration. Considering the effect of γ-irradiation, it decreased the Ce3+/Ce4+ ratio of 0.05CeGB and 0.3CeGB glasses while it slightly increased this ratio in 1.5CeGB glass.
(a) The XANES spectra of Ce:GB glasses before and (b) after γ-irradiation, compared to the unirradiated CeF3 and CeO2 standard compounds.
Results of the FTIR measurements for the 0CeGB and 1.5CeGB glasses before and after γ-ray irradiation in Fig. 3 indicate that the borate group was the main structural unit in glass network. The infrared vibration at 992 cm−1 corresponded to the B–O stretching vibration of tetrahedra BO4 units in tri-, tetra- and pentaborate27,43,44. While the B–O stretching of trigonal BO3 and tetrahedra BO4 units were attributed to the vibration around 1122 cm−127,43. The FTIR absorption around 1342 cm−1 was assigned to the B–O stretching vibration of the trigonal (BO3)3− units in meta-, pyro- and orthoborates27,43. The vibration centered at 2923 and 2852 cm−1 corresponded to the O–H stretching of hydroxyl OH− groups, while the broad band around 3288 cm−1 revealed to the vibration of OH− groups and B–OH linkage27,45. Before γ-irradiation, the vibration strength of these BO4, BO3, OH groups and B-OH linkage in 1.5CeGB glass were weaker than 0CeGB glass, indicating that the chemical groups in CeF3-doped glasses have poorer connectivity compared to the undoped glass. After irradiation, the γ-rays could break some chemical bonds in the glass network, resulting to the decrease in vibration strength of those chemical complexes. The infrared absorption by such complexes then reduced which caused the increment of FTIR transmittance after irradiation. The change in vibration strength of 1.5CeGB glass due to γ-rays damage was less than that of the 0CeGB glass.
The FTIR of 0CeGB and 1.5CeGB glass before/after γ-irradiation.
The transmission spectra of the Ce:GB glasses before γ-irradiation are shown in Fig. 4a. The unirradiated 0CeGB glass exhibited strong absorption in UV region with a transmission edge wavelength around 320 nm and the transmission spectra was shifted to longer wavelength with addition of CeF3 content. This red-shift of glasses influenced by CeF3 concentration were also found in several literatures18,34,35,38,46. Considering on the effect of γ-rays, the 0CeGB glass after irradiation obviously absorbed photons in UV and VIS regions, as shown in Fig. 4b. The γ-rays generated the color center that increased the absorption in both regions, especially in the VIS range.
(a) Transmission spectra of Ce:GB glasses before γ-irradiation41 and (b) after γ-irradiation, (c) The comparative transmission spectra and pictures before/after γ-irradiation of 0CeGB and (d) 1.5CeGB glass.
The increased absorption after irradiation is called “radiation-induced absorption” that can be considered from the change of transmittance before (T0) and after (T) irradiation at each wavelength by following relation47,
where αD is the radiation-induced absorption coefficient at each wavelength and x is the optical path length or thickness of sample. The αD were calculated in a range of VIS, 400–800 nm that the typical coefficient at 477 nm (αD-477) and the average coefficient (αD-ave) were shown in Table 2. The decrease of αD values represented the less effect of irradiation on glass with added CeF3 concentration, corresponding to the comparative transmission spectra and pictures of 0CeGB and 1.5CeGB glasses in Fig. 4c,d. The transmittance and color of 0CeGB glass changed significantly by irradiation, but they were very similar for 1.5CeGB glass.
The transmittance spectra were used to analyze the color parameters (L*, a* and b*) of glasses using the CIE 1976 L*a*b* Color Space (CIELAB)48 The values of CIELAB parameters are represented in Table 2. The magnitude of L* represents the brightness level, the positive/negative value of a* indicates the red/green approach and the positive/negative value of b* implies the yellow/blue approach of specimen. In glasses before γ-irradiation, more negative value of a* and more positive value of b* with increasing CeF3 content corresponded to the change of glass color becoming more greenish yellow, as can be seen in Fig. 1. After irradiation, γ-rays decreased the L*, changed the a* to be positive and increased the a*, resulting to the dark tone color of undoped 0CeGB glass. For CeF3-doped glasses after irradiation, the change of L* and b* tended to be less while the change of a* was fluctuating with addition of CeF3. To evaluate the total change of glass color that is damage from γ-rays, the color difference (∆Eab*) between glasses before and after irradiation were calculated by equation48,
where ∆L*, ∆a* and ∆b* is the difference values of such color parameters, before and after irradiation. The ∆Eab* value decreased with increment of CeF3 concentration corresponding to more similar color that the 1.50CeGb glass after irradiation came back to be bright and greenish yellow as same as itself before irradiation.
The refractive index (n) of the glasses are shown in Table 3. The value of n for unirradiated glasses increased as the amount of CeF3 increased. Considering the effect of γ-rays, the refractive index of irradiated glasses were higher than their unirradiated value. The difference in refractive index (Δn) before and after irradiation progressively decreased with increment of CeF3 content. All n values were used to calculate the molar refraction (Rm) and the molar polarizability (αm), respectively by following relations49,50,
where N is the Avogadro’s number. The αm parameter is the net electronic polarizability in glass that indicates the response of electrons to the electric field from incoming electromagnetic wave49,50, D-line light in this case. The variations of αm value influenced by the CeF3 concentration and γ-irradiation were in similar trend with the n value as shown in Table 3. This means the electrons in glasses were more sensed and the molecules were easily polarized to such electric field by the increment of CeF3 dopant and the γ-irradiation. The change of polarizability due to irradiation (Δαm) was smaller with adding CeF3 concentration.
The αD-ave, ∆Eab* and Δn value as a function of CeF3 concentration were plotted cooperatively in Fig. 5 which those parameters owned the similar behavior on variation of CeF3 content. This represents the ability of CeF3 that enhanced the radiation hardness on glass optical properties.
The average radiation-induced absorption coefficient (αD-ave), color difference (∆Eab*) and refractive index difference (Δn) of Ce:GB glasses.
The PL emission (solid line) and excitation (dash line) spectra under direct Ce3+ excitation of glasses before irradiation are shown in Fig. 6a. The luminescence intensity increased with increasing CeF3 concentration in the range of 0.00–0.30 mol%. The intensity decreased for CeF3 amounts larger than 0.30 mol% due to concentration quenching. The UV with 310 nm wavelength directly excited to the Ce3+ and promoted this ion from the ground 4f (2F5/2) to the excited 5d state. The Ce3+ then relaxed to the lowest vibrational 5d state via a non-radiative relaxation (NR) process, followed by the 5d → 4f (2F5/2) transition where a photon with 360 nm wavelength was emitted34,38,40. After irradiation, the PL spectra in Fig. 6b shows a similar peak position as the spectra before irradiation. Concentration quenching was also observed when the CeF3 doping concentration was more than 0.30 mol%. However, the luminescence intensity of the irradiated glasses decreased compared to the unirradiated ones. A clear evidence of intensity degradation is represented in the comparative spectra of 0.3CeGB glass in Fig. 6c. Moreover, an excitation peak around 275 nm of Gd3+ was also found and it overlapped on the left side of the Ce3+ excitation peak in those Fig. 6. The emission spectra of glasses under Gd3+ excitation were then studied and shown in Fig. 7a–c. The peak position, the influence of CeF3 concentration and γ-irradiation on emission intensity were similar with the spectra under direct Ce3+ excitation. Additionally, a small peak of Gd3+ emission under 6P7/2 → 8S7/2 transition was found at 312 nm wavelength. The strength of the Gd3+ emission peak was weakened with increasing CeF3 concentration because the excitation energy of Gd3+ was more transferred to Ce3+. The mechanism is as follows: the UV excitation with 275 nm excited the Gd3+ from 8S7/2 to 6I7/2 state. NR then took Gd3+ down to 6P7/2 level which was the intersection for next two separate routes. The first one was the 6P7/2 → 8S7/2 transition where Gd3+ emitted the photon with 312 nm. For the second route, the energy transferred from 6P7/2 state of Gd3+ to 5d state of Ce3+. After that, the 5d → 4f (2F5/2) transition of Ce3+ emitted the photon with 360 nm34,38,40. Furthermore, there was a probability that the excitation with 275 nm also directly excited to Ce3+ because its energy range of 5d state in glass is wide and overlaps with the 6I7/2 state of Gd3+. This appeared as the overlapping between the excitation peak of Gd3+ and Ce3+ in the PL spectra. The possible mechanisms about the energy transition of Ce3+ and Gd3+ in the PL spectra are represented in Fig. 8.
(a) The PL emission and excitation spectra under Ce3+ excitation of Ce:GB glasses before γ-irradiation and (b) after γ-irradiation, (c) the comparative PL spectra before and after γ-irradiation of 0.3CeGB glass.
(a) The PL emission spectra under Gd3+ excitation of Ce:GB glasses before γ-irradiation41 and (b) after γ-irradiation, (c) the comparative PL emission spectra before and after γ-irradiation of 0.3CeGB glass.
The possible energy level diagram for PL spectra of Ce:GB glasses.
The decay curves of Ce:GB glasses under 290 nm excitation before and after irradiation are shown in Fig. 9a,b, respectively. All decay curves were fitted well with a single exponential function. The decay time values before irradiation were in the range of 17.84–24.41 ns and their values after irradiation were between 21.25 and 27.18 ns. These short decay times in the order of tens of nanoseconds are the signature of Ce3+ luminescence under the 5d → 4f transition34,39,46. The decay time values increased with increasing CeF3 content in the range of 0.00–0.30 mol%, while they decreased for CeF3 concentration ranging from 0.30 to 1.50 mol%. This variation of decay time was similar with the change of PL intensity influenced by CeF3 amount. Moreover, it was found that the γ-irradiation caused an increment of decay time value in each glass.
(a) The decay curves under 290 nm excitation of Ce:GB glasses before γ-ray irradiation and (b) after γ-irradiation.
The RL spectra before and after γ-irradiation in Fig. 10 show a strong emission from Ce3+ around 381 nm wavelength. The incoming X-rays could initially interact to the glass host. The X-rays energy then transferred to luminescence center (Gd3+ or Ce3+). After that, Gd3+ could emit 312 nm luminescence but could not be detected in this experiment because of lower limit detection of spectrometer. There is also another possibility of Gd3+ transferred the energy to Ce3+ for luminescence under the 5d → 4f transition, similar process with the PL spectra. Additionally, the 5d–4f transition of Ce3+ could be occurred from this scintillation process. The RL intensity of glasses tended to quench for CeF3 concentrations that was greater than 0.30 mol%, like what was observed in the PL spectra. The γ-irradiation degraded the RL intensity of each glass which can be clearly observed in the comparative RL spectra of 0.3CeGB glass in Fig. 10c. The RL intensity of 0.3CeGB glass after irradiation decreased by 35% compared to its pre-irradiation intensity.
(a) The RL spectra of Ce:GB glasses before γ-irradiation41 and (b) after γ-ray irradiation, (c) the comparative RL spectrum before and after γ-ray irradiation of 0.3CeGB glass.
In this part, only the influence of CeF3 concentration on glasses before γ-irradiation are discussed and some explanations will be used continuously in the next subsection. The CeF3 dopant possibly acted as a glass modifier that created non-bridging oxygen (NBO) and disrupted the connectivity of borate groups in the Gd2O3-B2O3 glass network. Consequently, the FTIR vibration strengths of those borate complexes in 1.5CeGB were weaker than in 0CeGB glass. The vibration of OH- groups in FTIR were also reduced by CeF3 increment due to the reaction with F- ion as followed, 2OH− + 2F− → 2HF +in binary glass preparation as the glass O241,51. Since the transmission edge of host 0CeGB glass was overlapping to the 4f.-5d transition of Ce3+, the transmission spectra of CeF3-doped glasses then were red-shifted by more influence of this transition via increasing CeF3 dopant. This red-shift caused the change of CIELAB parameters in Table 2 and the observed change of color becoming more greenish yellow in Fig. 1. Additionally, there were reported that the addition of NBO could increase the glass optical basicity and consequently affected to the red-shift in absorption–transmittance spectra34,52,53,54. The electrons at NBO sites in glass are less tightly bound and can be easily oscillated by the electric field from incoming light, compared to electrons at bridging oxygen sites34,52. The polarizability of glass then increased by following number of NBO with addition of CeF3 content. Since the light was more sensed by the electrons, this light-electron interaction slowed down the speed of light (v) in glass resulting to the increment of refractive index by n = c/v relation with added CeF3 concentration.
The electronic configuration of Ce3+ ion is 4f1, which means that it has only one electron in the 4f shell to lose in order to have a more stable empty state. Therefore, Ce3+ can change to Ce4+ by losing one of its 4f. electron through the direct ionization, thermal ionization, or donation to hole by the process: Ce3+ + hole → Ce4+. On the other hand, a Ce4+ ion can accept an electron to form Ce3+ via the reaction: Ce4+ + electron → Ce3+38,41. This causes the coexistence of Ce3+ and Ce4+ ion in cerium doped materials, such as our glasses in this work. Since the electrons at NBO sites are less tightly bound, these electrons density could distribute and affect to the behavior of an 4f electron of Ce3+. The electrostatic pull between Ce3+ nucleus and its 4f orbital was weaken by such negative charges from NBO41,55,56. This increased the probability of Ce3+/Ce4+ ratio reduction with increasing of CeF3 content as observed in the XANES spectra. Concentration quenching was found in both the PL and RL spectra of glasses doped with CeF3 higher than 0.3 mol%. Quenching is due to the re-absorption of photons that are emitted by closely nearby Ce3+ neighbors. The shorter distance between Ce3+ ions and the dense ion distribution in glass provide this quenching effect, which also led to the reduction of decay time for glasses with more than 0.3 mol% of CeF3.
Considering the 0CeGB glass after irradiation, γ-rays could break some B–O–B and B–O–H linkages by following Eqs. (5) and (6), respectively.
Both “⋅ O –” and “– O ⋅ ” are the NBO, while the “≡ B ⋅ ” is the deformed borate complexes45. Generally, the NBO and deformed borate are the charge defect which naturally pre-exist in the unirradiated metal-oxide borate glasses, also in our Gd2O–B2O3 system, the γ-irradiation just increased the number of these complexes. The γ-rays could also ionize the chemical composition that generated the electron and hole in glass structure47. This hole and electron could separate and move to trap with those charge defects in glass. Hole could be trapped by negative charge of NBO to form the non-bridging oxygen hole center (NBOHC). The electron was probably trapped by positive charge of deformed borate, becoming to the boron electron center (BEC)45,57,58. However, there was reported that the BEC in borate glass was unstable for temperature above 120 K and its number dramatically decreased to be negligible at about 320 K59,60. Therefore, the main color center in our glasses after irradiation is NBOHC. This hole center is thought to absorb the photon around 3.8 eV (326 nm) and 2.6 eV (477 nm), that’s why the darkening and color change was obviously appeared in 0CeGB glass47,57,61,62. This corresponded to the high value of radiation-induced absorption coefficient at 477 nm and its average value in VIS range of this glass as shown in Table 2. The radiation-induced absorption coefficients in UV range lower than 400 nm were not analyzed due to the overlapping of 4f.-5d transition from Ce3+ on absorption of glass host. Since the irradiation possibly destroyed the B–O–B, B–O–H linkage and OH group shown in Eqs. (5) and (6), and disrupted the glass structure by formation of NBOHC, the FTIR vibration strength of 0CeGB glass then significantly decreased after irradiation. Moreover, the charge complexes such as the NBO and NBOHC created by γ-rays raised obviously the value of polarizability and refractive index in this glass.
For CeF3-doped glasses after irradiation, the electron donation from Ce3+ to hole inhibited the hole trapping at the charge defect site such as NBO38,47,61,63. Consequently, the number of NBOHC was decreased and the structure of 1.5CeGB glass was more conserved from the disruption than 0CeGB glass, as shown in FTIR spectra. The NBOHC reduction with the addition of CeF3 content also caused a decrease of those radiation damage parameters such as the radiation-induced absorption coefficient, the color difference, the change of polarizability and refractive index. Especially in 1.5CeGB glass, these values were close to zero which represented the highest radiation hardness.
The donation of an electron from Ce3+ to hole caused the reduction of Ce3+/Ce4+ ratio in 0.05CeGB and 0.3CeGB glass after irradiation, observed by XANE spectra. For 1.5CeGB glass, the large amount of CeF3 dopant created high number of pre-existed NBO in the glass network, and there were the electrons created by irradiation those could not trap to BEC because this center was unstable as previously mentioned. Some Ce4+ possibly accepted an electron from NBO and unstable BEC which changed this ion back to Ce3+. The Ce3+/Ce4+ ratio in 1.5CeGB glass therefore slightly increased by irradiation. The PL and RL luminescence intensity of CeF3-doped glasses decreased after irradiation due to the reduction of Ce3+/Ce4+ ratio. Since the absorption energy of defect (NBOHC at 3.8 eV) in UV region overlapped to the 4f–5d transition of Ce3+ in this glass, the UV excitation energy on decay time measurement was possibly trapped by defect, resulting to longer decay time after irradiation64.
From all results, the 0.3CeGB glass is a promising new glass scintillator, with the highest emission intensity among the glasses studied in this work, a relatively fast nanoseconds decay time and excellent radiation hardness.
Various properties of CeF3-doped Gd2O3-B2O3 glasses before and after γ-irradiation were comparatively investigated. XANES results show that the major and minor oxidation states of cerium ion in glasses were Ce3+ and Ce4+, respectively. Before irradiation, the analysis of glasses’ transparency, FTIR, refractive index and polarizability indicated that CeF3 degraded the connectivity and possibly created NBO in glass structure. This NBO caused the reduction of Ce3+/Ce4+ ratio, the red-shift in transmission spectra and the raise of refractive index with addition of CeF3 content. That red-shift also was influenced by 4f–5d transition of Ce3+ dopant. After irradiation, γ-rays damaged the glass structure, broke the chemical bond, and created color center in the borate network former. That center is NBOHC which absorbed photons in VIS region, resulting to the darkening and color change in glasses after irradiation. Moreover, the polarizability and refractive index of glasses were increased by the formation of NBO and NBOHC generated by irradiation. The addition of CeF3 concentration in glass relieved these irradiation effects. Due to the electron donation from Ce3+ to hole, number of NBOHC were annihilated. The radiation damage indicators such as the radiation-induced absorption coefficient, the color difference, the change of polarizability and refractive index then decreased in value with increasing CeF3 dopant. These results confirm the ability of CeF3 that enhances the radiation hardness of glass. The PL and RL intensity of CeF3-doped glasses decreased after irradiation due to the reduction of Ce3+/Ce4+ ratio via electron donation of Ce3+. The decay times of glasses after irradiation were longer, compared to them before irradiation because the excitation energy was possibly trapped by defect (NBOHC). The Gd2O3-B2O3 glass doped with 0.30 mol% of CeF3 exhibited the highest emission intensity, fast 24–27 ns decay time and owned the radiation hardness property, making it a promising new glass scintillator.
Yanagida, T. et al. Study of the correlation of scintillation decay and emission wavelength. Radiat. Meas. 55, 99–102 (2013).
Yanagida, T. Inorganic scintillating materials and scintillation detectors. Proc Jpn. Acad. Ser. B Phys. Biol. Sci. 94, 75–97 (2018).
Article ADS CAS PubMed PubMed Central Google Scholar
Arikawa, Y. et al.Pr3+-doped fluoro-oxide lithium glass as a scintillator for nuclear fusion diagnostics.Rev. Fr.Sci.Instrum.Rev. 80, 113504 (2009).
Article ADS PubMed CAS Google Scholar
Watanabe, K. et al. Pr or Ce-doped, fast-response and low-afterglow cross-section-enhanced scintillator with 6Li for down-scattered neutron originated from laser fusion. J. Cryst. Growth 362, 288–290 (2013).
Article ADS CAS Google Scholar
Yamanoi, K. et al. Luminescence properties of Nd3+ and Er3+ doped glasses in the VUV region. Opt. Mater. (Amst.) 35, 1962–1964 (2013).
Article ADS CAS Google Scholar
Olarinoye, I. O., Alomairy, S., Sriwunkum, C. & Al-Buriahi, M. S. Effect of Ag2O/V2O5 substitution on the radiation shielding ability of tellurite glass system via XCOM approach and FLUKA simulations. Phys. Scr. 96, 065308 (2021).
Al-Buriahi, M. S., Sriwunkum, C., Arslan, H., Tonguc, B. T. & Bourham, M. A. Investigation of barium borate glasses for radiation shielding applications. Appl. Phys. A Mater. Sci. Process. 126, 1 (2020).
Al-Buriahi, M. S., Bakhsh, E. M., Tonguc, B. & Khan, S. B. Mechanical and radiation shielding properties of tellurite glasses doped with ZnO and NiO. Ceram. Int. 46, 19078–19083 (2020).
Al-Buriahi, M. S., Singh, V. P., Alalawi, A., Sriwunkum, C. & Tonguc, B. T. Mechanical features and radiation shielding properties of TeO2–Ag2O-WO3 glasses. Ceram. Int. 46, 15464–15472 (2020).
Al-Buriahi, M. S. et al. Effect of chromium oxide on the physical, optical, and radiation shielding properties of lead sodium borate glasses. J. Non. Cryst. Solids 544, 120171 (2020).
Al-Buriahi, M. S. et al. Effect of Sb2O3 addition on radiation attenuation properties of tellurite glasses containing V2O5 and Nb2O5. Appl. Phys. A Mater. Sci. Process. 127, 1–12 (2021).
Hegazy, H. H. et al. The effects of TeO2 on polarizability, optical transmission, and photon/neutron attenuation properties of boro-zinc-tellurite glasses. J. Inorg. Organomet. Polym. Mater. 31, 2331–2338 (2021).
Al-Buriahi, M. S., Somaily, H. H., Alalawi, A. & Alraddadi, S. Polarizability, optical basicity, and photon attenuation properties of Ag2O–MoO3–V2O5–TeO2 glasses: The role of silver oxide. J. Inorg. Organomet. Polym. Mater. 31, 1047–1056 (2021).
Alzahrani, J. S. et al. Simulating the radiation shielding properties of TeO2–Na2O–TiO glass system using PHITS Monte Carlo code. Comput. Mater. Sci. 196, 110566 (2021).
Al-Buriahi, M. S. et al. Optical and radiation shielding studies on tellurite glass system containing ZnO and Na2O. Optik (Stuttg) 257, 168821 (2022).
Article ADS CAS Google Scholar
Edukondalu, A. et al. Synthesis, thermal, optical, mechanical and radiation-attenuation characteristics of borate glass system modified by Bi2O3/MgO. Appl. Phys. A Mater. Sci. Process. 128, 4 (2022).
Al-Buriahi, M. S., Alrowaili, Z. A., Alomairy, S., Olarinoye, I. O. & Mutuwong, C. Optical properties and radiation shielding competence of Bi/Te-BGe glass system containing B2O3 and GeO2. Optik (Stuttg) 257, 168883 (2022).
Article ADS CAS Google Scholar
Lai, Y. et al. Investigation of gamma-ray induced optical property changes in non-doped and Ce-doped lithium-rich oxide glass. Radiat. Phys. Chem. 179, 109272 (2021).
Wantana, N. et al. X-ray/proton and photoluminescence behaviors of Sm3+ doped high-density tungsten gadolinium borate scintillating glass. J. Alloy. Compd. 849, 156574 (2020).
Van Eijk, C. W. E. Inorganic scintillators for thermal neutron detection. Radiat. Meas. 38, 337–342 (2004).
Lee, G. H., Chang, Y. & Kim, T.-J. Properties and Possible Application Areas. Ultrasmall Lanthanide Oxide Nanoparticles for Biomedical Imaging and Therapy (Woodhead Publishing, 2014).
Xu, P. et al. Study on the sensitization of Gd3+ on Ce3+/Tb3+ co-doped GBS scintillating glass. J. Non. Cryst. Solids 481, 441–446 (2018).
Article ADS CAS Google Scholar
Wantana, N. et al. Radio, cathodo and photoluminescence investigations of high density WO3-Gd2O3-B2O3 glass doped with Tb3+. Radiat. Phys. Chem. 164, 108350 (2019).
Som, T. & Karmakar, B. Nephelauxetic effect of low phonon antimony oxide glass in absorption and photoluminescence of rare-earth ions. Spectrochim. Acta A Mol. Biomol. Spectrosc. 79, 1766–1782 (2011).
Article ADS CAS PubMed Google Scholar
Wantana, N. et al. Tunable orange, yellow and white emission of Pr3+-doped tungsten gadolinium borate glasses. J. Non. Cryst. Solids 554, 120603 (2021).
Taki, Y., Shinozaki, K., Honma, T., Dimitrov, V. & Komatsu, T. Electronic polarizability and interaction parameter of gadolinium tungsten borate glasses with high WO3content. J. Solid State Chem. 220, 191–197 (2014).
Article ADS CAS Google Scholar
Wantana, N. et al. High density tungsten gadolinium borate glasses doped with Eu3+ ion for photonic and scintillator applications. Radiat. Phys. Chem. 172, 108868 (2020).
Du, Y. et al. Luminescence properties of Ce3+-doped oxyfluoride aluminosilicate glass and glass ceramics. Opt. Mater. (Amst.) 89, 243–249 (2019).
Article ADS CAS Google Scholar
Boonsuth, R., Kaewjaeng, S., Kaewkhao, J., Lai, C. W. K. & Kothan, S. A study of the magnetic susceptibility on gadolinium calcium silicoborate glass. Mater. Today Proc. 5, 14892–14895 (2018).
Wantana, N. et al. Development of WO3-Gd2O3˗ B2O3 high density glasses doped with Dy3+ for photonics and scintillation materials application. Solid State Sci. 101, 106135 (2020).
Valiev, D. et al. Luminescence spectroscopy of scintillating glasses doped with Tb3+/Ce3+ with different concentrations of cerium under photo- and electron excitation. J. Lumin. 162, 128–133 (2015).
Wantana, N., Kaewjaeng, S., Kothan, S., Kim, H. J. & Kaewkhao, J. Energy transfer from Gd3+ to Sm3+ and luminescence characteristics of CaO–Gd2O3–SiO2–B2O3 scintillating glasses. J. Lumin. 181, 382–386 (2017).
Yanagida, T., Watanabe, K. & Fujimoto, Y. Comparative study of neutron and gamma-ray pulse shape discrimination of anthracene, stilbene, and p-terphenyl. Nucl. Instrum. Methods Phys. Res. Sect A Accel. Spectrom. Detect. Assoc. Equip. 784, 111–114 (2015).
Article ADS CAS Google Scholar
Wantana, N. et al. Ce3+ doped glass for radiation detection material. Ceram. Int. 44, S172–S176 (2018).
Park, J. M. et al. Luminescence properties of Ce3+ doped gadolinium-calcium-silicaborate glass scintillator. Radiat. Meas. 90, 166–169 (2016).
Kawano, N., Kawaguchi, N., Okada, G. & Fujimoto, Y. Scintillation and dosimetric properties of Sn-doped ZnO-SiO2-B2O3 glasses. J. Non. Cryst. Solids 482, 154–159 (2018).
Article ADS CAS Google Scholar
Ahmad, Z. et al. RADIO-OPTICAL response of cerium-doped lithium gadolinium bismuth borate glasses. J. Lumin. 224, 117341 (2020).
Rajaramakrishna, R., Kaewjaeng, S., Kaewkhao, J. & Kothan, S. Investigation of XANES study and energy transport phenomenon of Gd3+ to Ce3+ in CaO–SiO2–B2O3 glasses. Opt. Mater. (Amst) 102, 109826 (2020).
Zaman, F. et al. Scintillation and luminescence characteristics of Ce3+ doped in Li2O–Gd2O3–BaO–B2O3 scintillating glasses. Radiat. Phys. Chem. 130, 158–163 (2017).
Article ADS CAS Google Scholar
Rajaramakrishna, R. et al. Structural analysis and luminescence studies of Ce3+: Dy3+ co-doped calcium zinc gadolinium borate glasses using EXAFS. Radiat. Phys. Chem. 171, 108695 (2020).
Wantana, N. et al. Strong emission from Ce3+ doped gadolinium oxyfluoroborate scintillation glasses matrix. Radiat. Phys. Chem. 185, 109497 (2021).
Zu, C. K. et al. Effect of cerium on luminescence and irradiation resistance of Tb3+ doped silicate glasses. J. Alloy. Compd. 479, 294–298 (2009).
Sasi Kumar, M. V., Rajeswara Reddy, B., Babu, S., Balakrishna, A. & Ratnakaram, Y. C. Thermal, structural and spectroscopic properties of Pr3+-doped lead zinc borate glasses modified by alkali metal ions. J. Taibah Univ. Sci. 11, 593–604 (2017).
Kaewnuam, E., Wantana, N. & Kaewkhao, J. The study on Er3+ doped lithium bismuth aluminium borate glass for infrared medium applications. J. Phys. Conf. Ser. 1428, 012034 (2020).
Prabhu, N. S. et al. Structural and optical modifications in the BaO-ZnO-LiF-B2O3-Yb2O3 glass system after γ-irradiation. Materials (Basel) 14, 6955 (2021).
Article ADS CAS Google Scholar
Park, J. M. et al. Optical properties in the visible luminescence of SiO2:B2O3:CaO:GdF3 glass scintillators containing CeF3. J. Korean Phys. Soc. 71, 785–789 (2017).
Article ADS CAS Google Scholar
White, R. H. & Wirtenson, R. Radiation Induced Darkening of the Optical Elements in the Startracker Camera 33 (University of California, 1993).
Ohta, N. & Robertson, A. R. Colorimetry: Fundamentals and Applications (Wiley, 2006).
Vijayatha, D., Sujatha, B., Chandrashekaraiah, G., Reddy, C. N. & Reddy, N. S. Enhanced near-infrared luminescence at 10.7 μm of Nd3+ doped PbCl2–Li2B4O7 glasses for solid state laser and optical fiber amplifier applications. Opt. Mater. (Amst.) 111, 110543 (2021).
Rammah, Y. S., Sayyed, M. I., Abohaswa, A. S. & Tekin, H. O. FTIR, electronic polarizability and shielding parameters of B2O3 glasses doped with SnO2. Appl. Phys. A Mater. Sci. Process. 124, 1–9 (2018).
Luewarasirikul, N., Kim, H. J., Meejitpaisan, P. & Kaewkhao, J. White light emission of dysprosium doped lanthanum calcium phosphate oxide and oxyfluoride glasses. Opt. Mater. (Amst.) 66, 559–566 (2017).
Article ADS CAS Google Scholar
Bahadur, A., Dwivedi, Y. & Rai, S. B. Optical properties of cerium doped oxyfluoroborate glass. Spectrochim. Acta A Mol. Biomol. Spectrosc. 110, 400–403 (2013).
Article ADS CAS PubMed Google Scholar
Choi, S. Y. & Ryu, B. K. Optical, structural, and thermal properties of cerium-doped zinc borophosphate glasses. J. Nanosci. Nanotechnol. 15, 8756–8762 (2015).
Article CAS PubMed Google Scholar
Farouk, M., AbdEl-Maboud, A., Ibrahim, M., Ratep, A. & Kashif, I. Optical properties of Lead bismuth borate glasses doped with neodymium oxide. Spectrochim. Acta A Mol. Biomol. Spectrosc. 149, 338–342 (2015).
Article CAS PubMed Google Scholar
Chen, G. et al. The red-shift of ultraviolet spectra and the relation to optical basicity of Ce-doped alkali rare-earth phosphate glasses. J. Am. Ceram. Soc. 87, 1378–1380 (2004).
Bei, J. et al. Optical properties of Ce3+-doped oxide glasses and correlations with optical basicity. Mater. Res. Bull. 42, 1195–1200 (2007).
Shkrob, I. A. & Tadjikov, B. M. Magnetic resonance studies on radiation-induced point defects in mixed oxide glasses. I. Spin centers in B2O3 and alkali borate glasses. J. Non. Cryst. Solids 262, 6–34 (2000).
Article ADS CAS Google Scholar
Griscom, D. L. Electron spin resonance in glasses. J. Non-Cryst. Solids 40, 211–272 (1980).
Article ADS CAS Google Scholar
Pontuschka, W. M., Isotani, S. & Piccini, A. Optical and thermal bleaching of X-irradiated barium aluminoborate glasses. J. Am. Ceram. Soc. 70, 59–64 (1987).
Pontuschka, W. M., Kanashiro, L. S. & Courrol, L. C. Luminescence mechanisms for borate glasses: The role of local structural units. Glass Phys. Chem. 27, 37–47 (2001).
Pal Singh, G., Kaur, P., Kaur, S., Kaur, R. & Singh, D. P. Conversion of Ce3+ to Ce4+ ions after gamma ray irradiation on CeO2-PbO-B2O3 glasses. Phys. B Condens. Matter 408, 115–118 (2013).
Article ADS CAS Google Scholar
Kaur, R., Singh, S. & Pandey, O. P. Gamma ray irradiation effects on the optical properties of BaO-Na2O-B2O3-SiO2 glasses. J. Mol. Struct. 1048, 78–82 (2013).
Article ADS CAS Google Scholar
Wang, S. et al. Radiation hardness of Ce3+-doped heavy germanate glasses. Nucl. Instrum. Methods Phys. Res. Sect. Beam Interact. Mater. Atoms 201, 475–479 (2003).
Article ADS CAS Google Scholar
Kumar, V. & Luo, Z. A review on x-ray excited emission decay dynamics in inorganic scintillator materials. Photonics 8, 1–27 (2021).
This project is funded by National Research Council of Thailand (NRCT). J. Kaewkhao would like to thanks National Research Council of Thailand (NRCT) under Research Grants for Talented Mid-Career Researchers Project (Project number N41A640097). Thanks are also due to Thailand Science Research and Innovation (TSRI) for partially supporting this research.The authors gratefully acknowledge the Research Laboratory for Quantum Beam Science of the Osaka University Institute of Scientific and Industrial Research for assistance with gamma ray irradiation. M. Cadatal-Raduban gratefully acknowledges the Osaka University, Institute of Laser Engineering Collaborative Research Fund (2022B1-004) and the Catalyst: Seeding fund provided by the New Zealand Ministry of Business, Innovation and Employment and administered by the Royal Society Te Apārangi (CSG-MAU2003) for the financial support. This work was supported by JSPS KAKENHI Grant Number JP21K18909.
Physics Program, Faculty of Science and Technology, Muban Chombueng Rajabhat University, Ratchaburi, 70150, Thailand
Physics Program, Faculty of Science and Technology, Nakhon Pathom Rajabhat University, Nakhon Pathom, 73000, Thailand
N. Wantana, Y. Ruangtaweep & J. Kaewkhao
Center of Excellence in Glass Technology and Materials Science (CEGM), Nakhon Pathom Rajabhat University, Nakhon Pathom, 73000, Thailand
N. Wantana, Y. Ruangtaweep & J. Kaewkhao
Centre for Theoretical Chemistry and Physics, School of Natural Sciences, Massey University, Albany, Auckland, 0632, New Zealand
Institute of Laser Engineering, Osaka University, 2-6 Yamadaoka, Suita, Osaka, 565-0871, Japan
M. Cadatal-Raduban & K. Yamanoi
Department of Physics, Kyungpook National University, Daegu, 41566, South Korea
Synchrotron Light Research Institute (Public Organization), 111 University Avenue, Muang District, Nakhon Ratchasama, 30000, Thailand
You can also search for this author in PubMed Google Scholar
You can also search for this author in PubMed Google Scholar
You can also search for this author in PubMed Google Scholar
You can also search for this author in PubMed Google Scholar
You can also search for this author in PubMed Google Scholar
You can also search for this author in PubMed Google Scholar
You can also search for this author in PubMed Google Scholar
You can also search for this author in PubMed Google Scholar
E.K. analyzed the results, made the discussion, and edited the manuscript. N.W. measured and categorized the glasses properties. Y.R. prepared the glasses and found the optimum condition for synthesis. M.C.-R. and K.Y. provided the γ-irradiation on glasses and discussed its influence on the glasses properties. P.K. studied and analyzed the XANES spectra of glasses. H.J.K. and J.K. designed and gave the direction of research, including the consultation to develop this work.
The authors declare no competing interests.
Springer Nature remains neutral with regard to jurisdictional claims in published maps and institutional affiliations.
Open Access This article is licensed under a Creative Commons Attribution 4.0 International License, which permits use, sharing, adaptation, distribution and reproduction in any medium or format, as long as you give appropriate credit to the original author(s) and the source, provide a link to the Creative Commons licence, and indicate if changes were made. The images or other third party material in this article are included in the article's Creative Commons licence, unless indicated otherwise in a credit line to the material. If material is not included in the article's Creative Commons licence and your intended use is not permitted by statutory regulation or exceeds the permitted use, you will need to obtain permission directly from the copyright holder. To view a copy of this licence, visit http://creativecommons.org/licenses/by/4.0/.
Kaewnuam, E., Wantana, N., Ruangtaweep, Y. et al. The influence of CeF3 on radiation hardness and luminescence properties of Gd2O3–B2O3 glass scintillator. Sci Rep 12, 11059 (2022). https://doi.org/10.1038/s41598-022-14833-3
DOI: https://doi.org/10.1038/s41598-022-14833-3
Anyone you share the following link with will be able to read this content:
Sorry, a shareable link is not currently available for this article.
Provided by the Springer Nature SharedIt content-sharing initiative
By submitting a comment you agree to abide by our Terms and Community Guidelines. If you find something abusive or that does not comply with our terms or guidelines please flag it as inappropriate.
Scientific Reports (Sci Rep) ISSN 2045-2322 (online)

Holmium Sign up for the Nature Briefing newsletter — what matters in science, free to your inbox daily.